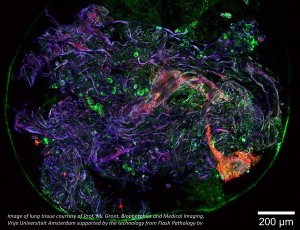
Finding the ideal ultra-fast laser source for a multiphoton imaging setup is not trivial. It is a fine balance between peak power, pulse energy and laser wavelength. In this white paper we discuss important laser parameters and the impact these laser parameters have on the quality of the results.
Background
Multiphoton and higher-order harmonics generation microscopy is particularly suited for high-resolution three-dimensional and live-cell imaging, where single photon techniques are limited by out-of-focus fluorescent background and penetration depth. Imaging techniques based on multiple photon interactions, such as two or three photon excitation and higher-order harmonics generation such as Second or Third Harmonic Generation (SHG or THG) imaging, have become very attractive tools in many biomedical research or clinical diagnosis applications, as they provide high contrast imaging capabilities with reduced tissue damage while not necessarily needing artificially induced fluorescent dyes.
For multiphoton excitations to occur, incident photons must arrive at the sample at the same time and place, in order to increase the probability of simultaneous multiphoton absorption events by a single fluorescent compound, which means that the efficiency in multiphoton excitation is strongly dependent on the peak intensity of the incident light during the pulse. Therefore, the shorter the pulse, the higher the peak intensity and the stronger the generated signal.
As a consequence of the nonlinear dependence on the light intensity in multiphoton microscopy and higherorder harmonics imaging, the signal is also only collected from within the plane of focus and the excitation light produces almost no out-of-focus emission. This resulting confined multiphoton excitation volume is especially suited for live-cell imaging, as it results in higher depth resolution and significantly less photodamage through the sample, which prolongs cell viability.
In addition, the longer wavelengths used in multiphoton imaging increases the penetration depth into the sample thanks to the lower scattering. Thus, multiphoton microscopy is often preferred over other techniques for experiments requiring deep penetration and imaging of living cells and tissue. Multiphoton excitation and higher order harmonics imaging require high peak intensities, which dictates the use of mode-locked lasers with ultrashort femtosecond pulses. To trigger a significant number of multiphoton absorption events, the photon density during the pulse must be orders of magnitude larger than in single photon absorption. Previously published results [1, 2] suggest that even shorter pulses than the commonly used pulse durations of 100-200 fs, enable lower cell lethality rates and allow for longer-term live-cell imaging. In this white paper we show that the use of sub 50 fs pulsed lasers produces very bright multiphoton and higher-order harmonics images while keeping the average laser power low, and that this presents a distinct advantage in extending live-cell imaging.
Imaging depth – longer wavelength?
One of the initial considerations in three-dimensional imaging is sample transparency at the selected laser wavelength. In most tissue, the penetration depth is limited by light-scattering due to the inhomogeneous nature of tissue. Light is scattered in anisotropic media, but longer wavelengths undergo less scattering than shorter wavelengths and therefore allow for deeper optical sectioning, a greater penetration depth and threedimensional imaging into thick samples. Light scattering (Rayleigh scattering) scales with 1/λ 4 , meaning that scattering is about 3 times less at 1 050 nm compared to at 800 nm. Although even longer NIR wavelengths (e.g. 1.3 µm or 1.7 µm) will further reduce light scattering effects, excellent deep tissue microscopy results can be obtained using wavelengths at around 1 050 nm. Absorption - shorter wavelengths? As shown in Figure 1, another challenge for penetration depth, and increasingly pronounced for longer NIR wavelengths, is absorption within the sample, especially by water. Exchanging water with D2O can help alleviate this to some degree, but the laser power will need to be reduced to avoid heating of the sample. The main chemical component of tissue is water and the absorption cross section of water in Figure 1, can be used to help identify a suitable excitation wavelength. As can be seen, the water absorption is lowest in the visible region. With 0.2% of the light absorbed per mm, there is also a local absorption minimum around 800 nm.
For three photon microscopy, however, a wavelength of 800 nm poses the problem that the signals emitted by samples are in the UV range (800/3 nm = 266 nm) which is a significant challenge in episcopic detection, where emitted light travels the same path as the excitation light. Emitted light from the sample passes through the same microscope objective that focuses the excitation light, which typically has limited UV transmission, especially below 350 nm. Therefore, for episcopic detection, a wavelength should be selected that is long enough to minimize light scattering and avoid poor UV transmission of third order signals, but short enough to minimize water absorption. In terms of scattering and absorption, using a center wavelength of around 1 050 nm therefore provides optimal conditions. Moreover, by using very short (sub 50) fs pulses, it is possible to achieve high signal to noise ratio and a deep penetration depth while keeping the average laser power down.
Avoid heating – lower the average power
Optical absorption of water at around 1 050 nm amounts to about 2% per mm of path length, which is considerable, but still orders of magnitude lower than at 1 700 nm. At 1 050 nm, with 100 mW average power focused into a field of view (FOV) of 0.5 x 0.5 mm2 , this leads to a local heat deposition of 8 mW per mm3 (0.02 * 100/0.5*0.5), which could cause a temperature increase of about 2 degrees per second (specific heat of water: 4.2 J.g-1 .K-1 ) [5]. For most tissues it would not create major problems when the temperature is raised by 1 degree, but for more sensitive tissue, such as lung tissue with trapped air, the sample may show significant “dynamics” due to the expansion of air. Also, prolonged exposure to a single area can cause thermal problems in all tissues at this power. With a smaller FOV, the average power is deposited on smaller volumes and the thermal heating increases and scales with the inverse of the FOV area [5]. Fortunately, at a length scale of 0.5 mm, thermal diffusion also takes place at a timescale of seconds (thermal diffusion constant of tissue is about 0.1 mm2 /s), which assists in removing heat. However, for high average powers it is inevitably a challenge to remove the generated heat fast enough to avoid causing thermal problems or damage in the tissue. Therefore, it is of great interest to achieve the best image quality at as low average laser powers as possible.
Why sub 50 fs pulses give brighter signals
Decreasing the pulse duration by a factor of four from 200 fs to 50 fs will result in a 4 times higher peak power (or peak intensity), for a given average power. Multiphoton and higher order harmonic signals depend non-linearly on the laser peak power. High intensity increases the likelihood of simultaneous multiphoton absorption events and the SHG and THG signals scale with the square and the cube of the incident light intensity, respectively. So, the corresponding signal increase is substantial for an increase in the laser peak power. Thus, ultrashort sub 50 fs pulses considerably improve multiphoton efficiency and higher-order harmonic generation efficiency and hence drastically increase image brightness.
To experimentally apply this relationship, it is essential to accurately determine the pulse duration at the sample. If dispersion in the microscope optics is not properly compensated for, the pulses will be stretched, and lower peak power will be measured at the sample. In such a case, the relationship between pulse duration and higher-order harmonics generation efficiency, cannot be accurately verified experimentally. To generate sufficient non-linear SHG and THG signals to achieve the required signal-to-noise ratio at average power levels that are gentle to the sample, sub 50 fs pulses and the use of dispersion pre-compensation is essential.
Figure 2: SHG and THG intensity vs. pulse duration Figure 3a) shows the third harmonic signal from a calibration grid using an average power of 4.7 mW on the sample, with the full bandwidth of the VALO Series femtosecond laser, yielding ~40 fs pulses.
Figure 3: Third harmonics of a calibration grid (Ibidi) with 50 micrometer squares. a) 4.7 mW, full spectrum short pulses (-50 fs; VALO series). B) 6 mW with laser spectrum imited to 10 nm bandwidth (~160 fs). c) Scaled up contrast for the 6 mW laser spectrum limited to 10 nm bandwidth (~160 fs).
In Figure 3b, the spectral bandwidth of the laser was restricted to 10 0 4 8 12 16 20. Figure 3a shows the third harmonic signal from a calibnm FWHM around 1 064 nm, resulting in ~160 fs pulses. The image in Figure 3a and 3b are identically scaled, but Figure 3b) shows no THG signal. Only after rescaling the lower THG signal from the longer ~160 fs pulses, was it possible to obtain an image above the experimental noise floor, as shown in Figure 3c. In this case, a 2.5 times higher average laser power was needed to achieve a signal to noise ratio comparable to that of THG signals obtained from shorter sub 50 fs pulses. In summary, ultrashort sub 50 fs pulses provide considerably higher pulse peak powers, which results in optimal signal-tonoise ratio images at much lower average power, which in turn reduces photobleaching, and extends cell viability.ration grid using an average power of 4.7 mW on the sample, with the full bandwidth of the VALO Series femtosecond laser, yielding ~40 fs pulses. In Figure 3b, the spectral bandwidth of the laser was restricted to 10
Intense pulses – advantages for THG
THG microscopy is a high resolution deep optical sectioning and label-free imaging technique, with reduced tissue damage and without the need for artificially induced fluorescent probes, but it requires higher peak powers and shorter pulses, while maintaining a low average laser power to extend cell viability. The emissions from endogenous biomolecules and the naturally occurring second-order harmonic signals have a chemical specificity for certain chromophores and are only produced in noncentrosymmetric molecules like collagen. THG signals are particularly much less chemically specific, and every spatial variation of the refractive index enables the generation of THG signals. As such, THG microscopy can be seen as a 3D equivalent of Phase Contrast Microscopy [6]. Especially, localized variations in refractive index to within half the laser focus dimension are particularly efficient at generating THG signals. Due to the Gouy phase shift in the focus, the laser field before and after the focus is 180 degrees out of phase. The same is true for all third order (and odd orders) signals, where signals have destructive interference and cancel out. Therefore, THG signals are not generated in a homogeneous medium, and a symmetry break is needed within the focus. Cellular and sub-cellular details can be observed due to this universal principle, some giving weak and
Figure 4: Mouse brain THG signals a) Large image mosaic,
Figure 4 b) Close-up image (200µm x 200 µm)
Molecules undergo several excitation/emission cycles before photobleaching, some may be more sensitive while others may perform several cycles before additional incident photons are unable to excite more molecules. In time-lapse microscopy, photodamage is one of the main limiting factors when observing living cells or tissue over extended periods of time. While phototoxicity is a function of molecular structure and the experimental environment, it is inevitably accelerated by constant exposure to a high average laser power. Thus, for live-cell imaging, sensitive to phototoxicity, the combined low average laser power and high peak power delivered by sub 50 fs pulses enhance cell viability and considerably extend imaging times. As shown in Table 1, comparing 50 fs to 200 fs, a laser with only one fourth the average power output is needed for the same peak power, which translates into longer imaging durations, while providing the same two-photon efficiency (TPEF). To achieve the lowest possible average power, lower repetition rate lasers are preferred, which also helps reduce photobleaching effects.
Table 1: Pulse duration, peak and average power comparison
Figure 5 shows THG imaging of myelin morphology and extended time-lapse acquisitions (over an hour) are performed without tissue damage, using the VALO Series sub 50 fs pulsed laser. The abaxonal and adaxonal myelin membrane are observed in the corpus callosum genu, and the role of sodium ion channels in the multiple sclerosis (MS) disease can be studied.
Figure 5: Time-lapse imaging of the induction of myelin-swelling during gradual high sodium perfusion, an indicator characterising the onset of multiple sclerosis (MS).
Sub 50 fs fiber lasers Fiber-based femtosecond lasers have become very attractive sources for various multiphoton and higherharmonics imaging applications due to their robustness, flexibility, ease-of-use, and low cost of ownership. The compact VALO Aalto and VALO Tidal lasers, deliver sub 50 fs pulses at a repetition rate of 30 MHz with pulse energies of 6.6 nJ and 66 nJ, and average powers of 200 mW and 2 W, respectively. They produce an alignmentfree high quality beam profile (typical M2
Figure 6: a) Typical pulse width and beam profile, b) typical optical spectrum of c) HÜBNER Photonics VALO Series femtosecond lasers.
Outlook
Multiphoton microscopy has immense potential for use in a large variety of applications including the development of future diagnostic screening systems. The VALO Series femtosecond lasers deliver pulses in the sub 50 fs regime and provide unprecedented multiphoton conversion efficiencies with intense peak powers. This in turn helps push frontiers in label-free multiphoton applications, especially in Third Harmonic Generation imaging since it enables extended live-cell imaging and time-lapse measurements with a high level of sensitivity.
Reference
s [1] Shuo Tang, Tatiana Krasieva, Zhongping Chen, Gabriel Tempea, Bruce Tromberg (2006), Effect of pulse duration on two-photon excited fluorescence and second harmonic generation in nonlinear optical microscopy, Journal of Biomedical Optics, 11(2). [2] Mira Sibai, Hussein Mehidine, Fanny Poulon, Ali Ibrahim, M. Juchaux, J. Pallud, A. Kudlinski, Darine Haidar (2018), The Impact of Compressed Femtosecond Laser Pulse Durations on Neuronal Tissue Used for TwoPhoton Excitation Through an Endoscope, Scientific Reports, 8:11124. [3] G.M Hale and M. R. Querry (1973), Optical Constants of Water in the 200-nm to 200-microm Wavelength Region, Appl. Opt., 12, 555-563. [4] Gert-Jan Bakker, Sarah Weischer , Júlia Ferrer Ortas, Judith Heidelin, Volker Andresen, Marcus Beutler, Peter Friedl (2022), Intravital DeepTumor Single-Beam 2-, 3- and 4-Photon Microscopy, eLife 11. [5] K. Giering , O. Minet , I. Lamprecht , G. Müller (1995), Review of thermal properties of biological tissues, SPIE PM, 25, 45-65. [6] Stephen A. Boppart, Sixian You, Lianhuang Li, Jianxin Chen, and Haohua Tu (2019), Simultaneous label-free autofluorescencemultiharmonic microscopy and beyond, cAPL Photon 4.
Acknowledgment This work has been conducted in collaboration with Flash Pathology and the Vrije Universiteit Amsterdam.
Written by Dr. Frank van Mourik Inventor & CTO, Flash Pathology, Dr. Marloes Groot Full Professor, Biophotonics and Medical Imaging, Faculty of Science, LaserLab, Vrije Universiteit Amsterdam, Niels Meijns PhD student, Anatomy & Neurosciences,Dr. Oliver Prochnow CEO, VALO Femtosecond lasers, HÜBNER Photonics, Dr. Wissam Nakhle Product Manager,-VALO Femtosecond lasers,- HÜBNER Photonics.